Love in a time of Prozac; how did our emotions evolve?
Seeing red: for happy faces, or snakes in the grass?
Gut feelings; are microbes in your intestines dictating your mood?
A cat hears a scuffling sound amongst the garbage. She stands in shadow, all senses poised.
A few moments pass; the rat emerges. In this alleyway, a light breeze carries her scent towards him. He sniffs the air, and looks in her direction. Two eyes blink, and then fixate upon him.
But he does not heed the warnings. A parasite in his brain, picked up from cat faeces, has immobilised his fear response. He scuttles out across the floor…
We are mostly bacteria. The microbes living on our skin, on the inner surfaces of our lung linings and through our gut outnumber our body cells ten to one. In fact, bacteria and other micro-organisms live in and around all multicellular life forms – from plants to people. This ‘microbiome’ is
an essential part of our biology and our health, and even influences how we (and all other animals) behave.
Microbes begin to colonise our gut from the moment of our birth. They help to pre-digest our food, making nutrients soluble and hence available for absorption through the gut wall. But the helpfulness of these bacteria doesn’t stop here.
The many small fatty acids and amino acids produced by bacterial fermentation act as signals inside our bodies. They initiate the development of the network of nerves in the gut wall; our ‘enteric nervous system’ (also known as our ‘second brain’). These nerves control the rhythmical contractions of the digestive canal, which operate independently of the central nervous system. Bacterial products also initiate, nourish and maintain the cells lining our gut.

A thin section of the small intestine wall, stained for CK20 protein (found in the mucosal lining).The lining of our intestines is a vast sensory surface, whose cells are nourished by fatty acids, vitamins and other com... morepounds produced by bacterial fermentation. The finger-like projections (called villi) visible in this section, form our main absorptive surface for nutrients, and sense the presence of both friendly bacteria and harmful pathogens (Image: Wikimedia Commons)
However the role of this microbial community goes beyond digestion.
– They out-compete harmful bacteria, ‘policing’ the gut and maintaining its pH.
– They present antigen signals (bacterial surface proteins) to our immune system, training us to recognise ‘friend’ from ‘foe’.
– They produce neurotransmitters and hormones, which are used directly and indirectly by the body. These include the cytokines and chemokines needed by immune cells to induce inflammation and fever responses and to target white blood cells into infected tissues. Bacteria produce precursors for 95% of our body’s serotonin (the ‘feel good’ brain chemical), and 50% of our dopamine.
Bacterial products affect the formation of connections between neurons (the synapses).

The enteric nervous system interacts with the central nervous system via the vagus nerve (the parasympathetic system) and the prevertebral ganglia (sympathetic nervous system). However if these nerve connections are sev... moreered, the enteric system will continue to function, integrating and resolving signals from the body and the environment. This network uses around 30 neurotransmitters, most of which are also found in the brain, and which include acetylcholine, dopamine and serotonin (Image: Wikimedia Commons)
Our ‘gut-brain axis’ is linked indirectly by chemicals the bacteria produce, which activate the immune system. Our mind and gut is also linked directly via the vagus nerve.
The vagus or ‘wandering’ nerve, our 10th cranial nerve, forms part of the parasympathetic (involuntary) nervous system. It is a ‘mixed nerve’, meaning it carries both sensory information about our body state back to the brain, including from the gut to the brain stem, and relaying messages from the brainstem and emotional centres to the body.
This information highway operates the ‘vagal reflex’, which relaxes the muscles around the stomach wall making space for our food, and also integrates the digestive process with our blood circulation, hormone system and emotional state.
This conversation between the digestive, immune, hormonal and nervous systems is essential for our healthy development. Mice raised under sterile conditions (so that their guts are free of bacteria) develop fewer connections between neurons, which results in retarded brain growth.
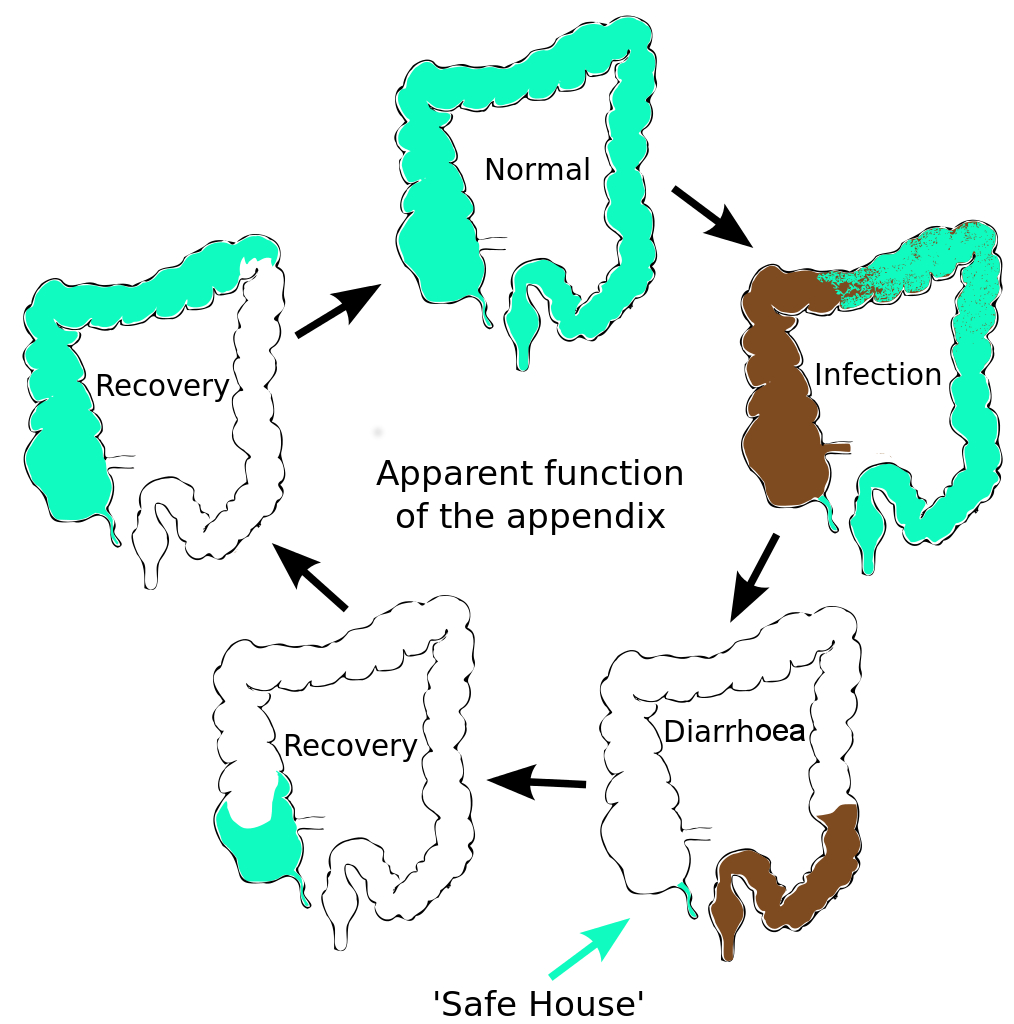
The human appendix is surrounded by copious amounts of immune tissue which particularly nurture and protect this sub-sample of our gut bacterial community. This creates a ‘safe house’ for a sample of our gut microfl... moreora. After an infection has triggered a diarrhoea response which expels the intestinal contents, the guts are recolonized by bacteria from this reservoir in the appendix. Charles Darwin first suggested that the human appendix is a relic of our evolution; a ‘vestige’ of a much larger mammalian caecum. (A caecum is a larger area of gut, containing bacteria, adapted in herbivores to digest large amounts of plant material). However this explanation doesn’t fit the facts. The appendix has evolved at least twice, arising independently in marsupials and placental mammals; an example of evolutionary convergence. This suggests that it has a current and selectable function (Image: Amended from Wikimedia Commons)
Gut microbes also influence our mood and emotions, affecting our behaviour. If intestinal bacteria from timid mice are used to populate the guts of a normally inquisitive mouse strain, these more adventurous mice become timid. Likewise, timid mice become adventurous when given the microflora from inquisitive mice.
Behavioural effects are also visible in humans. One of the first studies, conducted in France by Michaël Messaoudi and co-workers, found that drinking milk fermented with probiotic bacteria (versus non-fermented milk) lifted the mood of healthy human volunteers, reduced their blood cortisol levels (a hormone which increases during stress) and gave them a greater resilience against stimuli provoking symptoms of depression and anxiety.
Just as gut bacteria influence our health and mood, experiences that change our mood and behaviour in turn affect the composition of this microbial community. This shows that our gut microbiota are not autonomous, but act like a fully integrated body organ. This microbiotic organ monitors and responds to the food we eat, influences how we respond to our world, and connects with our body using messages sent in a common chemical ‘language’ . We speak about our ‘gut feelings’, usually unaware that this is much more than a metaphor.
It seems then, that our thoughts, feelings and behavioural choices affect our gut microbiome. As we ‘tell them’ how we feel (through the parasympathetic nervous system, immune system and hormones), they align their metabolic responses with this information. Their biochemical cues reinforce the body’s signal by releasing neurologically active chemicals that affect our mood. If our enteric nervous system really does deserve its title of the ‘second brain’, then the bacteria in our gut are mediating a connection that integrates the ‘thoughts of our guts’ with those of our mind.

Lab mouse, strain mg 3204. Laboratory mice are usually derived from the house mouse (Mus musculus). Mice are useful as a system to study many aspects of human health, thanks to having a high similarity with our genetic ... morecode, as well as the ability to thrive in a human-influenced environment. As with humans, many aspects of their development and behaviour are dependent upon a healthy community of gut microflora (Image: Wikimedia Commons)
Since we and all other animals are intimately associated with our gut bacterial ‘organ’, this raises interesting questions at the cellular level about where our physical boundaries really are.
Text copyright © 2015 Mags Leighton. All rights reserved.
References
Collins, S.M. and Bercik, P. (2013) Intestinal bacteria influence brain activity in healthy humans. Nature Reviews Gastroenterology & Herpetology 10, 326-327.
Cryan, J.F. and Dinan, T.G. (2012) Mind-altering micro-organisms; the impact of the gut microbiota on brain and behaviour. Nature Reviews Neuroscience 13, 701-712.
David, L.A. et al. (2014) Diet rapidly and reproducibly alters the gut microbiome. Nature 505, 559-566.
Diamond, B. et al. (2011) It takes guts to grow a brain. Bioessays 33, 588-591.
Faith, J.J. et al. (2011) Predicting a human gut microbiota's response to diet in gnotobiotic mice. Science 333, 101-104.
Forsythe, P. et al. (2010) Mood and gut feelings. Brain, Behaviour and Immunity 24, 9-16.
Frank, D.N. and Pace, N.R. (2008) Gastrointestinal microbiology enters the metagenomics era. Current Opinion in Gastroenterology 24, 4-10.
Heijtz, R.D. et al. (2011) Normal gut microbiota modulates brain development and behaviour. Proceedings of the National Academy of Sciences, USA 108, 3047–3052.
Jahan-Mihan, A. (2011) Dietary proteins as determinants of metabolic and physiologic functions of the gastrointestinal tract. Nutrients 3, 574-603.
Kurokawa. K. et al. (2007) Comparative metagenomics reveals commonly enriched gene sets in human gut microbiomes. DNA Research 14, 169-181.
Lee, W.J. and Brey, P.T. (2013) How microbes influence metazoan development: insights from history and Drosophila modelling of gut-microbe interactions. Annual Review of Cell and Developmental Biology 29, 571-592.
Lee, W.J. and Hase, K. (2014) Gut microbiota-generated metabolites in animal health and disease. Nature Chemical Biology 10, 416-424.
Lyer, L.M. et al. (2004) Evolution of cell-cell signalling in animals: did late horizontal gene transfer from bacteria have a role? Trends in Genetics 20, 292-299.
Messaoudi, M.et al. (2011) Assessment of psychotropic-like properties of a probiotic formulation (Lactobacillus helveticus R0052 and Bifidobacterium longum R0175) in rats and human subjects. British Journal of Nutrition 105,755-764.
Montiel-Castro, A.J. et al. (2013) The microbiota-gut-brain axis: neurobehavioural correlates, health and sociality. Frontiers in Integrative Neuroscience 7, article 70.
O’Hara, A.M. and Shanahan, F. (2006) The gut flora as a forgotten organ. EMBO Reports 7, 688-693.
Randal Bollinger, R. et al. (2007) Biofilms in the large bowel suggest an apparent function of the human vermiform appendix. Journal of Theoretical Biology 249, 826-831.
A sense of protection: is the immune system an extension of our minds?
Entering a body is much like entering a country.
At the border, agents verify the identity of those seeking to pass, and establish their status as ‘native’, a ‘naturalised resident’, or ‘alien’. Clarity about the identity of these travellers allows them to be appraised as ‘friend’ or ‘foe’.
The body of a nation operates best with safety measures in place. Its borders are a first line of surveillance that ascertains when to mobilise defences.
Border checks in some countries are more efficient; they deploy new technologies to implement higher orders of monitoring and identification.
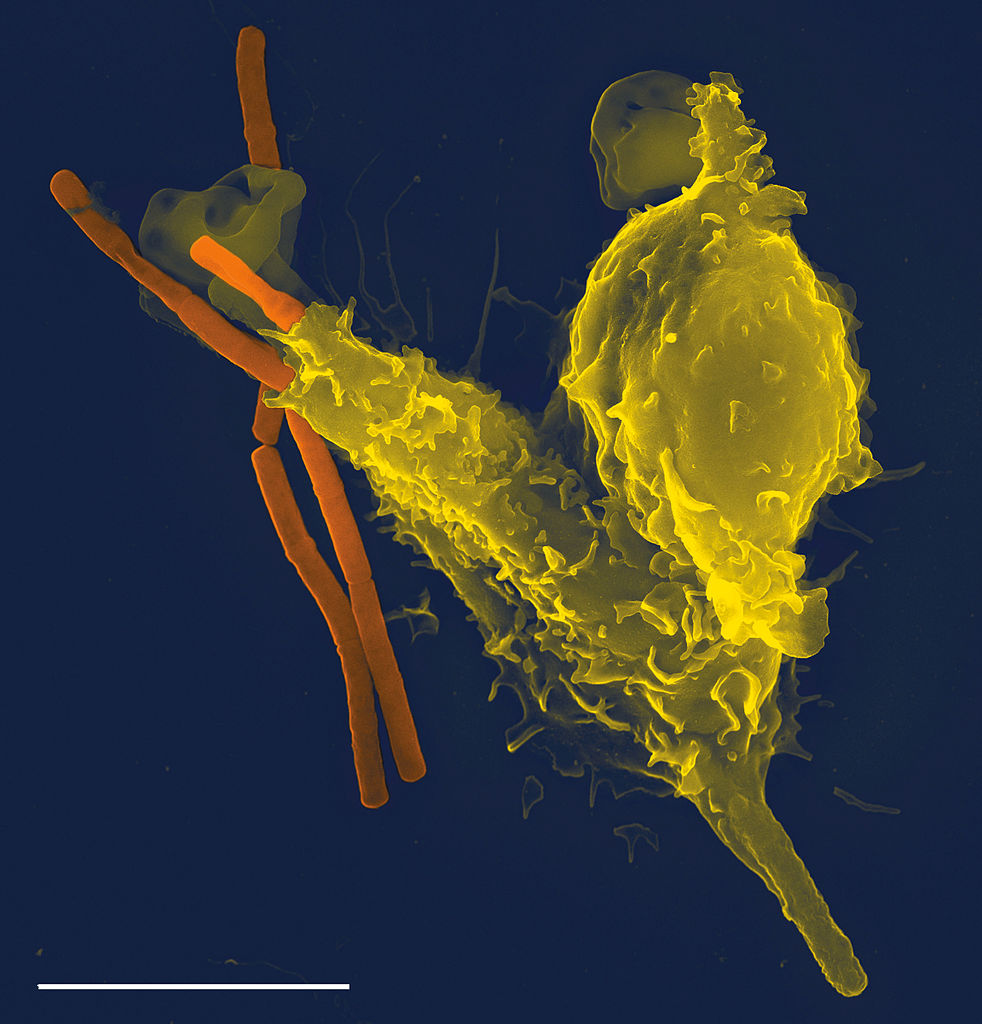
Scanning electron microscope image of a neutrophil, a cell from the innate immune system (yellow), engulfs anthrax bacteria (orange); scale bar = 5 micrometres.Body cells that experience stress, wounding, or sense the p... moreresence of bacteria, produce cytokines and other signals that trigger the innate immune response, and attract immune cells into the tissues.Some of these cells (‘phagocytes’) like this neutrophil, engulf and digest the invaders, whilst others (‘granulocytes’) secrete granules of cytokine, histamine and various types of oxygen free radicals, all of which enhance the inflammation response. Proteins in the blood, the ‘compliment system’, also condense onto and coat unwanted bacterial invaders and other particles. This targets them for destruction by phagocytes, or removal from the blood stream in the spleen (Image: Wikimedia Commons)
Typically we think of the immune system as a defence mechanism; the forces we mobilise to fight disease. Animals, plants, fungi and microbes all have a form of this type of defence. Even bacteria have a version of immunity, using enzymes that can react to and then digest viral proteins.
Immune responses in animal systems are thought of as having two levels of action.
Innate immunity
First, ‘innate immunity’ provides a spectrum of responses which use secreted proteins and dedicated cell types to target common components of a broad range of diseases.
Amongst animals, invertebrate bodies are relatively simple. In contrast, vertebrates have increased genetic and physical complexity, more sophisticated sensory perceptions and cognitive processing and, in some clades, enhanced social organisation and communication. This increased complexity is reflected in the vertebrate immune system.
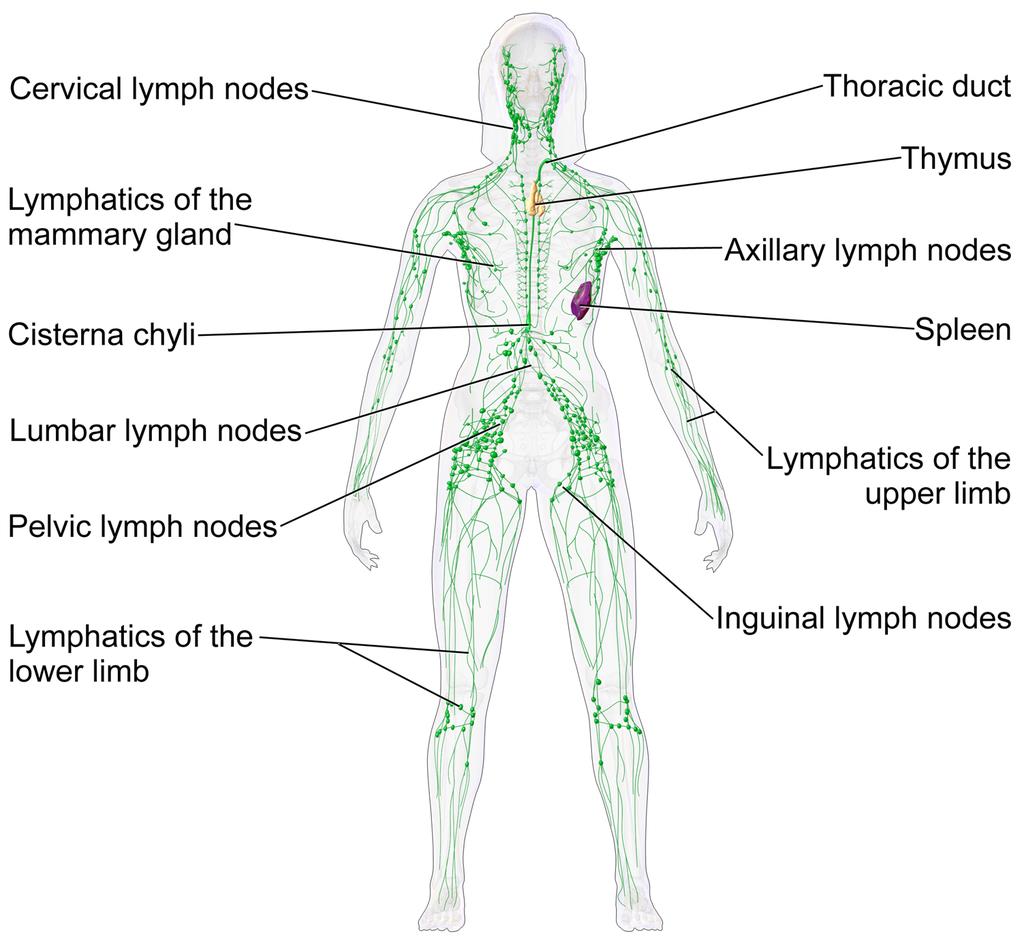
The lymphatic system of a human female. This network of vessels allows immune system cells rapid access to all areas of the body. Lymph vessels interconnect with the blood circulation at the heart and lymph nodes.Two or... moregans are particularly important in this network.• The thymus gland polices antibody-producing adaptive immune system cells, filtering out those which are too close to recognising native body proteins.• The spleen synthesises antibodies in its white pulp, and releases these proteins into the blood stream, where they bind to foreign proteins, such as those present on the surface of bacteria. This targets cells and other debris in the blood. The spleen removes antibody-coated bacteria and old red blood cells from the circulation. It also acts as a reservoir for monocytes, immune cells which later develop into phagocytic (non-specific engulfing) cells (Image: Wikimedia Commons)
Adaptive Immunity
The second form of immune response, known as ‘adaptive immunity’, refers to the ability to identify and target specific invaders. In vertebrates, this immune function first appeared in jawed fish.
In broad terms, it involves three phenomena.
– A new immunological organ (the thymus).
– A repertoire of specialised lymphocyte cells including T-cells (matured in and released from the thymus gland) and B-cells (released from the bone marrow), some of which produce antibodies.
– The production of a ‘self-identity’ signature, the ‘Major Histocompatibility Complex’ (MHC) proteins, in all body cells.
The community of bacteria associated with our gut, lungs and other surfaces comprise the first line of defence. This community of microbes (our ‘microbiome’) out-competes many harmful agents (pathogens).

Like the gut, our lung linings have a microbiome. Both ’friendly’ microbes and infective agents (here Streptococcus pneumoniae and Pseudomonas aeruginosa) produce proteins and other chemicals that act as signals. C... moreells lining our lungs act as signal receivers and recognise these chemicals, producing cytokines and other cues which relay messages to the immune system.(Image: Wikimedia Commons)
If our body cells become infected or damaged, they release stress signals such as oxygen and nitrogen free radicals and various peptides (short strings of amino acids) such as cytokines and defensins. These cues act as messages to the immune system, potentially triggering inflammation and other chemical defences which attract immune cells into the tissue by chemotaxis.
The microbial community also produces these immune-stimulating signals, along with brain-active chemicals (neurotransmitters). In addition, these signals are also generated, recognised by, and responded to by immune system cells.

Diagram of an antibody. These immune system proteins are a complex of four separate protein chains. In this diagram, blue regions are consistent whilst the yellow regions are variable. They are secreted into the blood s... moretream or gut. Adaptive immune cells use them as ‘antennae’. When this signal receiver encounters an antigen that is a good fit, this triggers an adaptive response. All proteins interact by shape. Antibodies have a recognition region which is highly variable. The strongest immune reactions provide a ‘best fit’ to this three-dimensional molecular jigsaw. (Image: Wikimedia Commons)
Our neurons and immune cells both respond to hormones within our bodies, since chemically these are close to neurotransmitters. This means that our brain, hormonal system, sensory nerves and extended microbial community all share a common chemical language with the innate immune system, present in all animals.
The greater the biochemical diversity of cell populations in a body, the more opportunities there are for bacteria or viruses to find novel ways of attacking these cells. For this reason, adaptive immune system cells themselves are vulnerable to certain types of disease. For instance AIDS (Acquired Immuno-Deficiency Syndrome), caused by the Human Immuno-deficiency Virus (HIV), specifically targets and infects the adaptive immune system’s T-cells, using the very mechanism that allows these cells to detect infection – their surface antibodies.
Potentially then, adaptive immunity provides a much more sophisticated and versatile immune mechanism. For vertebrates with their adaptive system to be ultimately more vulnerable to certain infections than the innate-only immunity of invertebrates seems bizarre and inefficient. Yet adaptive immune systems have evolved independently twice in vertebrates. This suggests that the adaptive mechanism must convey some survival advantages, but disease resistance may not be the primary role which has driven its evolution.
How does the system work, and what is the real purpose of adaptive immunity?
Adaptive mechanisms give our immune system a ‘memory’
In vertebrates, ‘adaptive immunity’ adds an additional higher-order function to the innate immune system by providing a means to both recognise and ‘remember’ specific diseases. To do this, they use a protein recognition system; these are the familiar antibodies.
These proteins have a shape-specific region which fits the shape of part of a foreign protein (an antigen) like a key in a lock. An antigen is a molecule that is capable of triggering an immune response; this can be from a foreign source (e.g. a virus) or produced by an unhealthy body cell, e.g. a cancer cell.

The adaptive immune system “adapts” to infections that get past our innate defences. Phagocytic macrophage (innate system) cells behave like amoebae, and can engulf and digest foreign bacteria. These ce... morells become ‘antigen presenting cells’ (APC), and displaying short pieces of bacterial protein (the antigen) on their cell surfaces, along with a self-signal, the ‘Major Histocompatibility Complex’ class II protein (MHC2). The MHC tells the immune system that this cell is a messenger, not an invader. These two proteins together activate the adaptive immune system.There are two types of adaptive response. First, T lymphocytes (T cells) display an antibody on their surface that recognises the foreign fragment on an APC. They then produce other surface proteins (here CD4+) that signal a change of identity, turning them into ‘‘helper’ cells. Helpers can provoke macrophages or B lymphocytes (B cells) to act. An activated B cell releases antibodies into the blood stream that ‘seek out’ and identify the invader. T cells can also become ‘killers’ that recognise and destroy infected body cells. (Image: Wikimedia Commons)
Adaptive immune cells called T-cells produce antibodies and ‘display’ them from their cell surfaces, using them as ‘antennae’ to detect invaders. Phagocytic (engulfing) innate immune cells can act as antigen presenting cells, displaying foreign protein fragments (antigens) on their surfaces. T-cells whose antibody ‘fits to’ this antigen can then be triggered to respond.
Triggered T-cells divide multiple times, so producing identical clones of themselves. This increases the magnitude of the body’s immune response to the infection. Activated T-cells relay the signal in turn to adaptive B-cells, triggering them to secrete large amounts of antibody proteins specific to this foreign antigen into the blood. These bind to the foreign antigens, coating the virus particles or foreign bacteria in antibodies. This targets the innate system’s macrophages to ‘engulf and destroy’.
Immune system cells interact with each other in a similar way to nerve cells
The association between the antigen presenting cell and the activating T-cell is very close, and the trigger mechanism transmits a one-way signal between the cells. This is very like how a nerve synapse transmits a signal to the next nerve. It is therefore entirely reasonable to call it an ‘immunological synapse’.
This between-cell interaction, which here involves a specific recognition between the immune cells, arises from an ancient, general mechanism of between-cell communication that allows signal-transmitting contacts to form. Activated T-cells initially show pulses of electrical activity, produced by a release of calcium ions (which carry an electrostatic charge) into the cell matrix (cytoplasm).
Synapses are stable contacts that form between two distinct cells that allow for information transfer through directed secretion. Synapses between neurons in the brain form relatively stable contacts, although these remain ‘plastic’, able to be recruited into new neural networks and adopted for new purposes.
In the immune system, ‘synapse’ formation between for example an antigen presenting cell and a T-cell, provokes the T-cell to initiate a signal relay transmitted to other cells which produces a range of specific ‘actions’. These responses, resulting in defences that target the specific foreign antigen, are produced by cells further downstream of this initial ‘synapse’.

When a patient is first vaccinated, the adaptive immune system raises a response against the foreign antigens. These cells persist at a high level for several weeks, then the antibody level and effector T-cell activity ... moregradually declines. When a patient is re-vaccinated, a stronger response results. Selection favours T-cells with small differences in antibody binding that give a stronger reaction. These highly specific clones remain in the serum and lymphatic tissues and provide protective immunity against reinfection by the same agent. This gives a longer term immunological memory; later reinfection leads to a rapid increase in antibody production and effector T cell activity, which often prevents the disease from being apparent (Image: Wikimedia Commons)
Vaccinations activate our body ‘memory’
By cloning and maintaining a population of cells with disease-specific antibodies, our immune system ‘remembers’ how it responded to the disease. A second exposure produces a refinement of the accuracy of the recognition mechanism, and raises a population of ‘memory T-cells’ that persist in the body for much longer. This is the basis of vaccination. Exposing our immune systems to proteins from the infective agent, via an injection, allows us to develop resistance in a controlled, safe way. Our bodies raise a population of immune cells that ‘remember’ how to recognise these foreign proteins. If we later meet the disease, these ‘memory cells’ activate, enabling us to more quickly overcome the infection and contract only a mild version of the disease, or perhaps experience no symptoms at all.
These mechanisms suggest that adaptive immunity should provide enhanced resistance to diseases beyond what the innate response alone can deliver. In practice, however, invertebrates seem to be vulnerable to fewer diseases than we are. This may be a result of their simpler and less diverse profile of cell and tissue types.

Diagram of an HIV particle. Using cell surface antibodies as an infective agent identification mechanism means that immune cells can in turn become ‘visible’ to these pathogens. The Human Immunodeficiency Virus (HIV... more) which causes Acquired Immuno-defficiency Syndrome (AIDS) specifically identifies T-cells. This virus is a master of disguise; it uses a similar gene shuffling mechanism as are used to produce variable proteins on the surface of its viral capsule. This changeability makes the virus seem to always be something new – this is the reason that the immune system has difficulty recognising and neutralising it, and also is why the virus has been so difficult to target using a vaccine. The interaction between an infection and the host immune system is usually like a predator prey relationship. The immune system is the ‘predator’, chasing down to consume the infection (the ‘prey). In the case of HIV, these roles are unclear. Is the virus pursing the immune cell, or is the immune cell pursuing the virus? (Image: Wikimedia Commons)
Why would vertebrates evolve this expanded complexity in their immune system?
Every living thing, including plants and bacteria, possess an immune system of some kind. What we recognise as innate immunity is found in plants and also throughout the animal kingdom. Adaptive immunity raises the degree of complexity. Significantly, it has evolved twice independently within the vertebrates; in jawed vertebrates (sharks to humans) and also in the more ‘primitive’ jawless clades. Certain invertebrate groups (e.g. snails and insects), and indeed also bacteria, have a quasi-adaptive system, using specific, cross-reactive molecules, although these systems are not as sophisticated as that found in the vertebrates.
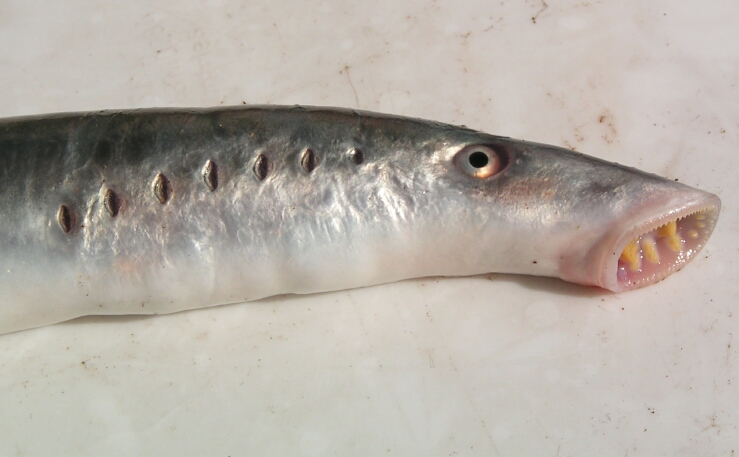
A river lamprey (Lampetra fluviatilis) from the German North Sea. The immune systems of this and other jawless fish such as the hagfish have been found to contain adaptive cells which act in a similar way to T- and B- l... moreymphocytes of the jawed vertebrates. Here the membrane proteins acting as signal receivers are different, based on receptors containing leucine-rich motifs. As in their jawed cousins, the immune responses of lampreys produce a clonal increase of specific disease-detecting cells (Image: Wikimedia Commons)
Studies in the jawless fish, lamprey and hagfish, show that a very different form of adaptive system has arisen than is found in jawed vertebrates, co-opted from a different set of genes and proteins. In contrast, their immune molecules include proteins with repeated sections in the chain which have multiple residues of the amino acid leucine (termed ‘Leucine Rich Repeats’, or LRRs). A form of LRR-based adaptive immunity is also found in plants.
Jawed vertebrate adaptive immunity however is rather more developed than that of the jawless fish. Jens Rolff makes two intriguing suggestions as to what may have driven its evolution.
i. Vertebrate bodies are larger and more complex than invertebrates, providing a new set of potential habitats for parasites. Rolf suggests that an evolutionary ‘arms race’ may have occurred between vertebrates and a group of parasitic Platyhelminthes, which include tapeworms and liver flukes. These parasites began to diversify after the evolutionary divergence between jawed and jawless fish.
The surface of these parasites is highly resistant to our immune defences, suggesting that they co-evolved in tandem with an immune-competent host. The increasingly more aggressive and targeted immune response required from the host to expel these parasites may have selected for the direct recognition mechanism and targeted responses of adaptive immunity. This theory also implies that the adaptive components of the immune system originally evolved in association with the gut.
ii. Vertebrates have higher metabolic rates than invertebrates. This allows for greater rates of activity and access to a wider range of ecological habitats. Higher energy bodies require more sophisticated brains and nervous systems, and have higher maintenance demands.

Scheme showing a DNA ligase-I enzyme (in color) repairing a break in DNA. DNA damage, caused by environmental factors and normal metabolic processes, occurs at a rate of 1,000 to 1,000,000 molecular lesions per cell per... more day. Without maintenance to mend such breaks, cells can malfunction, die, or become cancerous. Ligases catalyse the crucial step of joining the breaks in the spiralling DNA strand. To do so they require energy, in the form of either Adenosine triphosphate (ATP) or Nicotinamide adenine dinucleotide (NAD+) as a cofactor (Image: Wikimedia Commons)
Rolff suggests that the adaptive immune system is part of the normal homeostasis of a higher energy metabolism. Such regimes also produce higher concentrations of oxygen free radicals and other stress-associated compounds which can damage DNA and cause cancers. High energy vertebrate bodies therefore require a means of self-monitoring. The adaptive immune system may provide just such a set of ‘internal eyes and ears’.
How does the adaptive immune system function in vertebrates?
Our immune system discerns ‘friend’ from ‘foe’, ‘self’ from ‘non-self’ and monitors the status and identity of our own body cells. The adaptive system provides specific ways to identify and monitor foreign and native body cells.
An immunological synapse relay is triggered when for example a T-cell antibody recognises a foreign protein on an ‘antigen presenting cell’. This and other types of immunological synapse require a class of proteins related to antibodies; the ‘Major Histocompatibility Complex’ (MHC) proteins.
Vertebrates produce and display MHC proteins on their cell surfaces. During an infection, if a virus or microbial pathogen enters a body cell, it becomes invisible to the immune system. Infection, however, alters the health of the cell, reducing its surface MHC production. Reduced or absent MHC triggers ‘Natural Killer’ (NK) cells to form an immunological synapse with them, this time triggering the destruction of the infected body cell, and helping to reduce the spread of the disease.

As a cell is first infected by a microbial pathogen, (as in this diagram), it quickly mobilises its protein-dismantling enzymes (the ‘proteasome’) to digest the foreign proteins into short fragments (peptides). Spec... moreialised pores (‘Transport of Alien Protein’ or TAP channels) capture the fragments and shunt them into the endoplasmic reticulum, where the cell assembles its own proteins. Here the fragments are bound to ‘Major Histocompatibility Complex’ class 1 (MHC I) proteins. These are transported to the cell surface where they protrude like a beacon, presenting the foreign protein to the innate immune system’s ‘Natural Killer’ cells (Image: Wikimedia Commons)
MHC proteins also act as antigen presenting molecules. At an early stage of infection, the cell’s own defences may digest some of the invading pathogen’s proteins. These foreign antigens can be bound by MHC molecules, and presented on the cell surface. Again, this triggers NK cells to target this body cell for destruction.
Antigen-presenting phagocytic immune cells also do this using a different class of MHC protein, which avoids them activating the NK cells.
Recent research has also shown an unexpected role for MHC proteins in neuronal plasticity. Brain neurons require MHC class I molecules to establish new neural networks. Along with a range of other immune system receptors, these molecules are critical for the construction of new connections and the remodelling of synapses. This suggests that the transmission of a signal across the neural synapse and the immunological synapse are likely using the same mechanism.
What does this suggest as the primary role of adaptive immunity?

The ‘molecules of emotion’ with their effects. The mood effects that result from their deficiency in italics. These neurotransmitters are the same chemical language as is used by cells of the immune system (Image: W... moreikimedia Commons)
Candace Pert’s work demonstrated that immune cells both respond to and produce the same neurotransmitter signals as brain neurons. She initially found opiate receptors from the brain in immune system cells. Opiates are drugs that trigger the body’s responses to endorphins, the body’s natural ‘feel good’ chemicals. Pert showed that receptors for neurotransmitters which affect our mood; the ‘molecules of emotion’ including b -endorphins and serotonin, are present in immune system cells. Immune cells also manufacture these same chemicals.
Immune cells are everywhere in the body. Brain-based phenomena such as depression impact the function of the immune system such that our level of disease resistance rises and falls with our moods. In at least chemical terms, these cells are operating in conjunction with our neural network. Pert’s results reveal that through the immune system, our minds occupy the full sensory space of our bodies, and are not limited to our brains. By the same route, immune cells convey the chemical implications of our emotional state into every cell in our body. This means that the way we think has a direct impact on our health.
Adaptive immune cells recognise stress signals, neurotransmitters, and foreign proteins. Foreign antigen recognition activates the immunological synapse, and triggers the adaptive immune response. Recent research has shown that the MHC proteins, essential for this response, are also involved in the functioning of nerve synapses in the brain. This implies that the two systems are really both part of the same ‘neural super-organ’.
This ‘organ’ has flexibility as to where it focusses its attention. Release of cytokines and other stress signals draw immune cells into tissues where their surveillance is most required. This roving internal sense organ has been referred to by Enzo Ottavani and Claudio Franceschi as an ‘immune-mobile brain’ whose ‘eyes and ears’ are our adaptive immune cells.

Poster, emphasising the importance of surveillance, issued by the office for Emergency Management in the United Kingdom during the Second World War (Image: Wikimedia Commons)
This surveillance system cross-reacts with and can mutually influence the body’s hormonal system and gut microbiome. Edwin Blalock has suggests a primary role for the immune system as a sense organ; truly our ‘sixth sense’, which is able to detect stimuli not recognised by the central or peripheral nervous system. This information, the chemical language of the body’s bacterial community, is translated into a cognitively accessible form by the antennae of the adaptive immune system.
This provides a picture of the brain and immune system as forming an integrated ‘body-mind’. This system’s sensory perceptions, thoughts, emotions and behavioural responses impact the physical body at the biochemical level. This ‘embodiment’ idea has also become apparent through recent insights into the actions of mirror neurons and the neural basis of human language.
Having a conversation in the same language synchronises our minds. The brain and immune system translate this into hormonal and other chemical cues of emotion.
This suggests that our immune system is one part – a subconscious part – of our overall consciousness. This consciousness is integrated into our ‘body-mind’. Through sharing our thoughts with others, our awareness operates beyond the body at a higher level, and as we do this, we connect at the level of our chemistry.
Conclusions
- Innate immunity is general, and present in all cells in some form, from bacteria to humans.
- Adaptive immunity, where the organism raises a specifically cross-reacting chemical response to an infection, has evolved multiple times in multicellular life forms, including twice in vertebrates. Characteristics of adaptive immune systems in vertebrates are the appearance of cross-reacting proteins (antibodies) produced by specialised immune cells, a self-identifier (the major histocompatibility complex) in all body cells, and a thymus gland, which is a new organ within the lymphatic system.
- The driver of immune system evolution in vertebrates is not primarily defence. Factors that have driven the evolution of immune complexity in the vertebrate system (relative to invertebrates) may include (i) evolutionary ‘arms races’ with gut parasites, and (ii) an increased need to monitor the metabolic by-products of the higher energy vertebrate body.
- Immune complexity seems to be linked to the complexity of the overall organism, and fulfils a self-surveillance role. The increased complexity of vertebrate systems has in fact made them vulnerable to a wider range of infections than invertebrates.
- Vertebrate immune cells, neurons, and body cells all share a common chemical language; a language also used by the associated microbes that live on and around the vertebrate body (the microbiota).
- Immune cells form synapses, using a similar between-cell communication apparatus that neurons use to form their synapses. However the immune synapses are more transient, and this component of the body’s relay network is able to migrate to tissues where it is needed, augmenting the body’s surveillance in this area.
- Brain-body communication is two-way. The immune system integrates our thoughts and emotional state with our body, as well as informing the mind of the status of the body’s cells and tissues.
Text copyright © 2015 Mags Leighton. All rights reserved.
References
Abedon, S.T. (2012) Bacterial ‘immunity’ against bacteriophages. Bacteriophage 2, 50–54.
Bellinger, D.L. and Lorton, D. (2014) Autonomic regulation of cellular immune function. Autonomic Neuroscience 182, 15-41.
Bhalla, A.K. (1989) Hormones and the immune response. Annals of the Rheumatic Diseases 48, 1-6.
Bhat, R. and Steinman, L. (2009) Innate and adaptive autoimmunity directed to the central nervous system. Neuron 64, 123-132.
Blalock, J.E. (2005) The immune system as the Sixth Sense. Journal of Internal Medicine 257, 126-138.
Blalock, J.E. (2002) Harnessing a neural-immune circuit to control inflammation and shock. The Journal of Experimental Medicine 195, F25-F28.
Boulanger, L.M. (2009) Immune proteins in brain development and synaptic plasticity. Neuron 64, 93-109.
Brenneman, D.E. et al. (1988) Neuronal cell killing by the envelope protein of HIV and its prevention by vasoactive intestinal peptide. Nature 335, 639-642.
Bromley, S.K. et al. (2001) The immunological synapse. Annual Review of Immunology 19, 375-396.
Carr, D.J. et al. (1989) Hormones common to the neuroendocrine and immune systems. Drug Design and Delivery 4, 187-195.
Chacon, M.A. and Boulanger, L.M. (2013) MHC class I protein is expressed by neurons and neural progenitors in mid-gestation mouse brain. Molecular and Cellular Neuroscience 52, 117-127.
Chapman, R.C. et al. (2008) Pain and stress in a systems perspective; reciprocal neural, endocrine and immune interactions. The Journal of Pain 9, 122-145.
Cooper, E.L. (2010) Evolution of immune systems from self-not self to danger to artificial immune systems (AIS). Physics of Life Reviews 7, 55-78.
Elenkov, I.J. et al. (2000) The sympathetic nerve – an integrative interface between two super-systems; the brain and the immune system. Pharmacological Reviews 52, 595-638.
Goebel, M.U. et al. (2002) Behavioral conditioning of immunosuppression is possible in humans. FASEB Journal 16, 1869-1873.
Herbein, G. and Varin, A. (2010) The macrophage in HIV-1 infection; from activation to deactivation? Retrovirology 7, 33.
Malagoli, D. and Ottaviani, E. (2010) Life is a huge compromise; is the complexity of the vertebrate immune-neuroendocrine system an advantage or the price to pay? Comparative Biochemistry and Physiology, A 155, 134-138.
Ottavani, E. et al. (1988) The neuro-immunological interface in an evolutionary perspective; the dynamic relationship between effector and recognition systems. Frontiers in Bioscience 3, 431-435.
Ottovani, E. et al. (2007) Common evolutionary origin of the immune and neuroendocrine systems; from morphological and functional evidence to in silico approaches. Trends in Immunology 28, 497-502.
Ottaviani, E. and Franceschi, C. (1996) The neuroimmunology of stress from invertebrates to man. Progress in Neurobiology48, 421-40. Erratum in: Progress in Neurobiology 49, 285.
Pert, C. (1997) Molecules of Emotion; Why You Feel the Way You Feel. Schribner.
Pert, C. and Marriot, N. (2006) Everything You Need to Know to Feel Go(o)d. Hay House.
Polianova, M.T. et al. (2005) Chemokine receptor-5 (CCR5) is a receptor for the HIV entry inhibitor peptide T (DAPTA). Antiviral Research67, 83-92.
Pollitica, M. et al. (2007) Profound anti-HIV-1 activity of DAPTA in monocytes/macrophages and inhibition of CRR5-mediated apoptosis in neuronal cells. Antiviral Chemistry and Chemotherapy 18, 285-295.
Quan, N. and Banks, W.A. (2007) Brain-immune communication pathways. Brain, Behaviour and Immunity 21, 727-735.
Raberg, L. et al. (2002) Basal metabolic rate and the evolution of the adaptive immune system. Proceedings of The Royal Society of London, B 269, 817-821.
Rolff, J. (2007) Why did the acquired immune system of vertebrates evolve? Developmental and Comparative Immunology 31,476-482.
Rosi, S. et al. (2005) Chemokine receptor 5 antagonist D-ala-peptide T-amide reduces microglia and astrocyte activation within the hippocampus in a neuroinflammatory rat model of Alzheimer’s disease. Neuroscience 134, 671-676.
Ruff, M.R. et al. (2003) Update on D-ala-peptide T-amide (DAPTA); a viral entry inhibitor that blocks CRR5 chemokine receptors. Current HIV Research 1, 51-67.
Salles, A. et al. (2013) Barcoding T cell calcium response diversity with methods for automated and accurate analysis of cell signals (MAAACS). PLoS Computational Biology 9, e1003245.
Shatz, C.A. (2009) MHC class I: an unexpected role in neuronal plasticity. Neuron 64, 40-45.
Smith, E.M. and Blalock, J.E. (1988) A molecular basis for interactions between the immune and neuroendocrine systems. International Journal of Neuroscience38, 455-64.
Wahl, S.M. et al. (2006) HIV accomplices and adversaries in macrophage infection. Journal of Leukocyte Biology 80, 973-983.
Weigent, D.A. et al. (1990) Bidirectional communication between the neuroendocrine and immune systems. Common hormones and hormone receptors. Annals of the New York Academy of Sciences579, 17-27.
Wrona, D. (2005) Neural–immune interactions; an integrative view of the bidirectional relationship between the brain and the immune systems. Journal of Neuroimmunology 172, 38-58.
Yong, V.W. and Rivest, S. (2009) Taking advantage of the systemic immune system to cure brain diseases. Neuron 64, 55-60.
Yuan, S. et al. (2014) Amphioxus as a model for investigating evolution of the vertebrate immune system. Developmental & Comparative Immunology (in press).
What’s so different about human speech?
Upon leaving the island, Odysseus is warned that storms lie ahead. His route home to Ithaca passes the sirens; monsters whose beautiful, haunting voices lure sailors to their deaths.
He sets a course and explains his intention to the crew. At his command they fasten him to the mast, seal their ears with wax, and prepare for their encounter.
They reach treacherous waters. The siren song reaches into Odysseus’ mind, resonating with his deepest longings. The storm rages within. He struggles, but his bindings, the result of his clear intention, secure him tightly to the mast.
Forced to stand still and listen, he finds that he starts to hear the voices for what they really are; the empty fears of his own soul. He relinquishes his fight and hears the voice at his own still centre. The storm calms.
The crew notice that he has returned to his senses. They cut him loose.
He is indeed a wise and worthy captain.

This ancient footprint, first made in soft mud, is an index which shows us the passing of an three-toed Theropod dinosaur. Denver, Colorado (Image: Wikimedia Commons)
Speaking involves transmitting and interpreting intentional signs, some of which are also used in the instinctual communications of animals. These signals are of three kinds: 1. An index physically shows the presence of something, e.g. wolves tracking their prey by scent.
2. An ‘icon’ resembles the thing it stands for, like a photograph or a painting. Dolphins, apes and elephants recognise their own reflection; we assume that they interpret this two-dimensional image as representing their three-dimensional physical selves.
3. A symbol associates an unrelated form with a meaning. Our words are symbols, linking an idea with unique sound-and-movement sequences. They do not resemble the things they represent.

The ‘Union Jack’, a symbol of Great Britain since the union of Great Britain and Ireland in 1801. It is made up of three other flag symbols; the Cross of St George for England (insert, top), St Andrew’s Saltaire f... moreor Scotland (centre), and for Northern Ireland, St Patricks Saltire (below) (Image: Wikimedia Commons)
Symbolism is almost unknown amongst animals, with a few rare exceptions. A stereotypical form of symbol is the ‘waggle dance’ of honeybees.
Although chimpanzees can be taught to use some sign gestures, they do not naturally communicate using symbols. In contrast, we use our symbolic language intentionally.
Our uses of speech are unique. We revisit our memories, order our thoughts and make future plans. With a destination in mind, we can listen for our ‘inner voice’, map out our route, take a stand against the storm of inner and outer distractions, and find our way home.
How is our speech unique?

Normal speech is already multi-channel; our words are accompanied by the musicality of our speaking, and our facial expressions and other physical gestures transmit many layers and levels of complex meaning. Writing is ... moreanother mode of communicating our language. Social media transmits our language into virtual worlds. The online social networking service Facebook commissioned ‘Facebook Man’ to commemorate their 150 millionth user(Image: Wikimedia Commons)
Aspects of our language ability are found in other animals, but the way we have combined and developed these traits is uniquely human.
1. We use any available channel.
Most human languages use vocal speech. Under circumstances where speaking is not possible, we find other ways, e.g. sign languages and Morse code.
2. We build our words from parts that gain meaning as they are combined
Most of the syllables we use to build words lack meaning on their own. Combining them together (as in English) or adding tonal shifts (as in Chinese) creates words.
3. We code our words with meanings, making them into symbols

The chimpanzee (Pan troglodytes) known as Washoe (1965-2007) was the first non-human animal to be taught American sign language. She lived from birth with a human family, and was taught around 350 sign words. It was rep... moreorted that upon seeing a swan, Washoe signed “water” and “bird”. Chimpanzees are capable of learning simple symbols. However Washoe did not make the transition to combining these symbols together into new meanings (Image: Wikimedia commons)
Symbols are ‘displaced’, i.e. they do not need to resemble the thing they represent. Our words symbolise ideas, experiences and things.
4. We combine these symbols to make new meanings.
We build words into phrases and stories, use these to revisit and share our memories, combine them into new forms, and communicate this information to others in various ways. Combining different symbols brings us a new understanding, which changes how we respond.
Look at this painting. As you do, consider what feelings it provokes.

‘Wheatfield with crows’ by Vincent Van Gogh, 1890. (Image; wikimedia commons)
It is, of course, by Vincent Van Gogh. As you may know, his choices of colour and subject material were a personal symbolic code. He often used vibrant yellows, considering this colour to represent happiness.
His doctor noted that during his many attacks of epilepsy, anxiety and depression, Van Gogh tried to poison himself by swallowing paint and other substances.
As a consequence, he may have ingested significant amounts of toxic ‘chrome yellow’, which contains lead(II) chromate (PbCrO4).
Now consider this statement.
“This is the last picture that Van Gogh painted before he killed himself” (John Berger 1972, p28)
Look again at the picture.
What do you feel this time?

‘Wheatfield with crows’ by Vincent Van Gogh, 1890. (Image; wikimedia commons)
Certainly our response has changed, though it is difficult to articulate precisely what is different. The image now seems to illustrate this sentence. Its symbolic content has altered for us. This example shows how combining two types of information –an image and text- can change the meaning it symbolises.
Some animals can be trained to recognise simple symbols. The psychologist Irene Pepperberg taught her African Grey parrot ‘Alex’ to count; he learned to use numbers as symbols, and could identify quantities of up to 6 items.

An African Grey Parrot (Psittacus erithacus). Professor Irene Pepperberg’s parrot, Alex, learned basic grammar, could identify objects by name, and could count (Image: Wikimedia Commons)
5. The order in which we combine symbols defines their meaning
We put word symbols together into phrases, sentences, descriptions, sayings, stories, poems, documents, manuals, plays, oaths, promises, parodies, plays, pantomimes….
The ordering of words follow rules (grammar and syntax). Animals such as dogs and dolphins show some form of syntactical ability, but there is no evidence that they are on the verge of using what we understand as language. The order of words shows us their relationship, allowing us to understand how they are interacting. We change the order of our words and phrases to change the meaning we wish to communicate.
For instance; this makes sense.
‘Jane asked Simon to give these flowers to you.’
This doesn’t quite fit our normal understanding of reality…
‘These flowers asked Simon to give Jane to you.’
This works, but the meaning has changed.
‘Simon asked you to give these flowers to Jane’
However grammar is not enough . The words in combination need to ‘make sense’ for us to understand the meaning the speaker wishes to communicate.
What does this enable us to say?
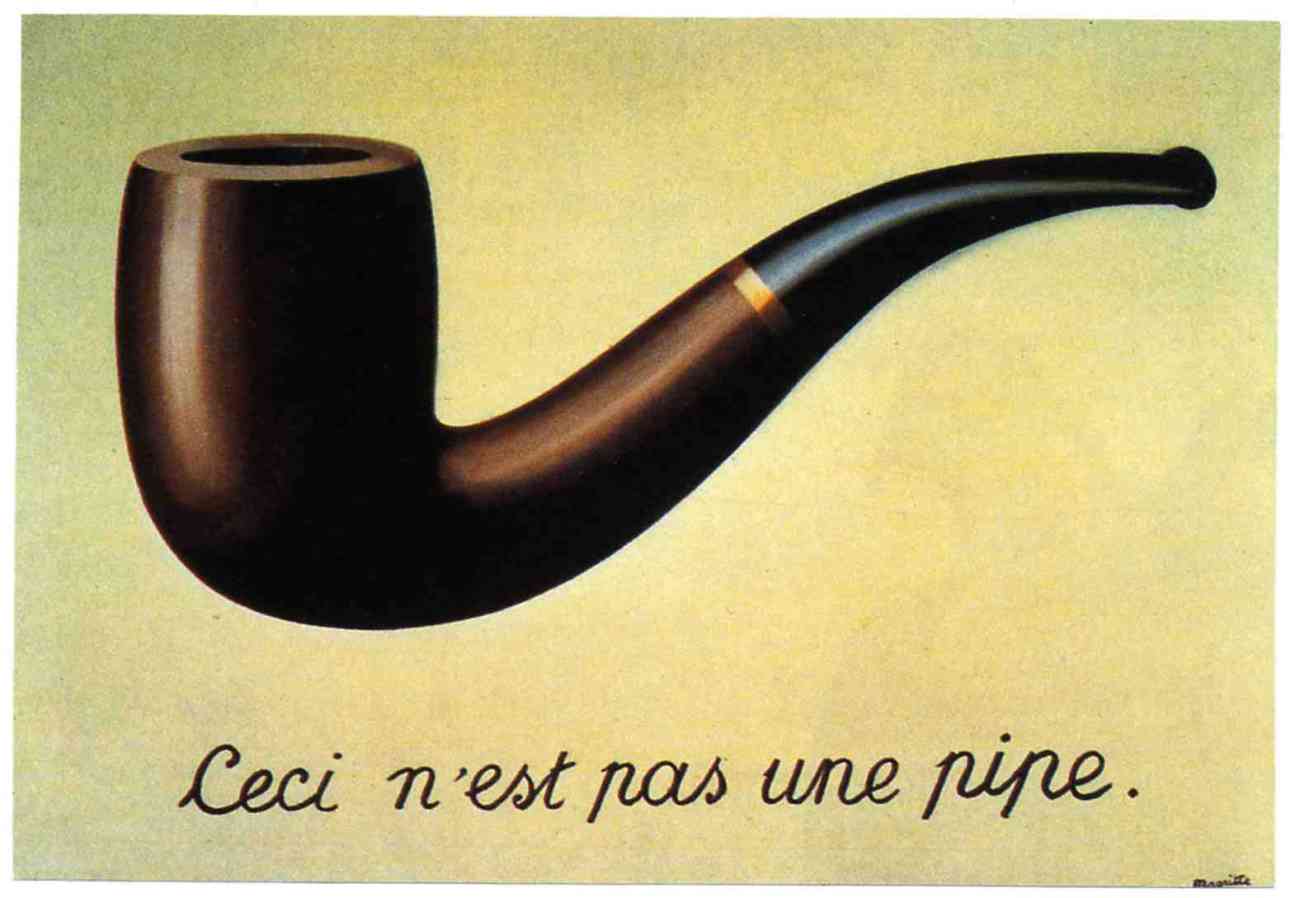
‘The treachery of images’ by Belgian surrealist painter, Rene Magritte (1928-9).Much of Magritte’s work explored the combination of words and images, and the way that this challenges the meaning that we understand... more from the components on their own. This combination of words and image have been deliberately chosen so that they contradict each other.What the artist says is true. However, it isn’t a pipe! It is a two-dimensional representation of a pipe (Image: Wikimedia Commons)
When we make new combinations of words, or add words to a visual signal such as a gesture, we create a new meaning.
We can add adjectives to a description, add qualifiers, combine phrases into a sentence, and make statements one after the other so that our listener associates these ideas. This process is known as ‘recursion’, a linguistic term borrowed from mathematics.
Our ideas about time vary between cultures, but we all mentally ‘time travel’ by revisiting our memories. For instance, the scent of something can evoke a memory that transports us back into an earlier event; suddenly we experience again the emotions and sensations we felt at that time. Putting our current selves into the past memory, or imagining a future scenario and inserting ourselves into that story, is a form of recursion.
Memory allows us to link speaking and listening with the meanings of our words. Our language is well structured to easily express recursive ideas. This shows us that our thinking uses recursion.
Why are we able to do this?

An illustration by Randolph Caldecott (1887) for ‘the House that Jack Built’. This traditional British nursery rhyme uses recursion to build up a cumulative tale. The sentence is expanded by adding to one end (end r... moreecursion). Each addition adds an increasingly emphatic meaning to the final item of the sentence (i.e. the house that Jack built) (Image: Wikimedia Commons). One final version of combined phrases ends like this;This is the horse and the hound and the hornThat belonged to the farmer sowing his cornThat kept the cock that crowed in the mornThat woke the priest all shaven and shornThat married the man all tattered and tornThat kissed the maiden all forlornThat milked the cow with the crumpled hornThat tossed the dog that worried the catThat killed the rat that ate the maltThat lay in the house that Jack built.
Our thinking capacity, through which we learn and remember, means that we can copy and learn to use language. Although some brain regions appear specialised for roles in memory and language, our ‘language function’ uses our entire brain, and cannot be dissociated from our minds.
Our ‘language brain’ includes the ‘basal ganglia’; these are neurons which connect the outer cortex and thalamus with lower brain regions.
We need this connectedness to coordinate movements in our fingers, to understand the relationships between words that are inferred by their order in our phrases, and to solve abstracted (theoretical) problems. This network interacts with ‘mirror neurons’ which allow us to relate to and decode the posture, speech and emotional cues of others.
The basal ganglia that influence our speech also regulate the muscles controlling our posture. Standing is therefore more than just balancing on two legs; it is a whole body activity and requires much finer muscle control than walking on all fours. It also frees the hands, which allows us to manipulate tools. Lieberman suggests that it is the fine motor control required to maintain our upright posture which pre-adapted our ancestors for manipulating hand tools as well as the tongue, lips and other structures that make speech possible. This upright posture is linked with a remodelling of our breathing apparatus, giving us more control over our larynx.
Philip Lieberman’s work with people suffering from Parkinson’s’ disease suggests that it is the ability to remember that makes speaking possible. Parkinson’s patients have degraded nerve circuits in their basal ganglia, so these patients have short term memory problems and difficulties with balancing and making precise finger movements. They also struggle with understanding and using metaphors and longer word sequences. This suggests that when we speak we are using the circuitry for sorting and remembering movement sequences, irrespective of whether these are producing words or actions.

Our posture has remodelled the evolution of our entire physiology from breathing to childbirth. It frees the hands, allowing us to perform delicate and precise sequences of tasks. Selection for the ability to precisely ... moresequence our manual motor skills may have provided our ancestors the means to better sequence their thoughts (Image: Wikimedia Commons)
The basal ganglia that influence our speech also regulate the muscles controlling our posture. Standing is therefore more than just balancing on two legs; it is a whole body activity and requires finer muscle control that walking on all fours. It also frees the hands, which allows us to manipulate tools.
Lieberman suggests that it is the fine motor control required to maintain our upright posture which pre-adapted our ancestors for manipulating hand tools as well as the tongue, lips and other structures that make speech possible. This upright posture is linked with a remodelling of our breathing apparatus, giving us more control over our larynx.
The nerve networks that control our limbs and voices are linked across all vertebrates. Our basic ‘walking instinct’ initially activates Central Pattern Generator circuits driving movement in all four limbs. These are the same neural outputs that control our lips, tongue and throat.
Conclusions: What does this say about our language?

Captain Odysseus stands upright against the mast. This posture is distinct to our species, and has many implications for our speech, language and other actions
(Image: Wikimedia commons)
- Our hominin ancestors evolved to use symbolic words and stories as a code to store and share memories, develop new skills and ideas, and coordinate their intentions and actions with their tribe.
- When we revisit our memories or ‘reword’ our experiences into new sequences, we remodel the past, and project our thoughts into the future.
- The control we have over our vocal sounds is linked with our neural circuits for movement. The ability to balance ideas and manipulate our tongues is linked to our ability to stand upright, balance on two feet and manipulate tools with our hands.
- Language, then, is a cultural tool that allows us to order our thoughts, go beyond our instincts, share our intentions, and choose our own story.
Text copyright © 2015 Mags Leighton. All rights reserved.
References
Berger J (1972) ‘Ways of Seeing’ Penguin books Ltd, London, UK
BickertonD and Szathmáry E (2011) ‘Confrontational scavenging as a possible source for language and cooperation’ BMC Evolutionary Biology 11:261 doi:10.1186/1471-2148-11-261
Corballis MC (2007) ‘The uniqueness of human recursive thinking’ American Scientist Volume 95 (3), May 2007, Pages 240-248
Corballis, M.C.(2007) ‘Recursion, language, and starlings’ Cognitive Science 31(4) 697-704
Everett D (2008) ‘Don’t sleep, there are snakes: Life and language in the Amazonian jungle’ Pantheon Books, New York, NY (2008)
Everett, D (2012) ‘Language: the cultural tool’ Profile Books Ltd, London, UK
Gentner TQ et al (2006) ‘Recursive syntactic pattern learning by song birds’ Nature, 440;1204–1207
Riddles in code; is there a gene for language?
‘I have…’
Words are like genes; on their own they are not very powerful. But apply them with others in the right phrase, at the right time and with the right emphasis, and they can change everything.
‘I have a dream…’
Genes are coded information. They are like the words of a language, and can be combined into a story which tells us who we are.
The stories we choose to tell are powerful; they can change who we become, and also change the people with whom we share them.
‘I have a dream today!’
Language is a means for coding and passing on information, but it is cultural, and definitely non-genetic. Nevertheless, for our speech capacity to have evolved, our ancestors must have had a body equipped to make speech sounds, along with the mental capacity to generate and process this language ‘behaviour’. Our body’s development is orchestrated through the actions of relevant genes. If the physical aspects of language ultimately have a genetic basis, this implies that speech must derive, at least in part, from the actions of our genes.
The hunt for genes involved with language led researchers at the University of Oxford to investigate an extended family (known as family KE). Some family members had problems with their speech. The pattern of their symptoms suggested that they inherited these difficulties as a ‘dominant’ character, and through a single gene locus.

The FOXP2 gene encodes for the ‘Forkhead-Box Protein-2’; a transcription factor. This is a type of protein that interacts with DNA (shown here as a pair of brown spiral ladders), and influences which genes are turne... mored on in the cell, and which remain silent. This diagram shows two Forkhead box proteins, which associate with each other when active. This bends the DNA strand and makes critical areas of the genetic code more accessible (Image: Wikimedia Commons)
Discovery of another unrelated patient with the same symptoms confirmed that the condition was linked to a gene known as FOXP2 (short for ‘Forkhead Box Protein-2’). This locus encodes a ‘transcription factor’; a protein that influences the activation of many other genes. FOXP2 was subsequently dubbed ‘the gene for language’. Is that correct?
Not really. FOXP2 affects a range of processes, not just speech. The mutation which inactivates the gene causes difficulties in controlling muscles of the face and tongue, problems with compiling words into sentences, and a reduced understanding of language. Neuroimaging studies showed that these patients have reduced nerve activity in the basal ganglia region of the brain. Their symptoms are similar to some of the problems seen in patients with debilitating diseases such as Parkinson’s and Broca’s Aphasia; these conditions also show impairment of the basal ganglia.

Genes code for proteins by using a 3-letter alphabet of adenine, thymine, guanine and cytosine (abbreviated to A, T, G and C). These nucletodes are knwn as ‘bases’ (are alkaline in solution) and make matched pairs w... morehich form the ‘rungs of the ladder’ of the DNA helix. Substituting one base for another (as happens in many mutations) can change the amino acid sequence of the protein a gene encodes. Changes may make no impact on survival, allowing the DNA sequence to alter over time. Changes that affect critical sections of the protein (e.g. an enzyme’s active site), or critical proteins like FOXP2, are rare (Image: Wikimedia Commons)
Genes provide the code to build proteins. Proteins are assembled from this coding template (the famous triplets) as a sequence of amino acids, strung together initially like the carriages of a train and then folded into their finished form. The amino acid sequences of the FOXP2 protein show very few differences across all vertebrate groups. This strong conservation of sequence suggests that this protein fulfils critical roles for these organisms. In mice, chimpanzees and birds, FOXP2 has been shown to be required for the healthy development of the brain and lungs. Reduced levels of the protein affect motor skills learning in mice and vocal imitation in song birds.
The human and chimpanzee forms of FOXP2 protein differ by only two amino acids. We also share one of these changes with bats. Not only that, but there is only one amino acid difference between FOXP2 from chimpanzees and mice. These differences might look trivial but they are probably significant. FOXP2 has evolved faster in bats than any other mammal, hinting at a possible role for this protein in echolocation.

Mouse brain slice, showing neurons from the somatosensory cortex (20X magnification) producing green fluorescent protein (GFP). Projections (dendrites) extend upwards towards the pial surface from the teardrop-shaped ce... morell bodies. Humanised Foxp2 in mice causes longer dendrites to form on specific brain nerve cells, lengthens the recovery time needed by some neurons after firing, and increases the readiness of these neurons to make new connections with other nerves (synaptic plasticity). The degree of synaptic plasticity indicates how efficiently neurons code and process information (Image: Wikimedia Commons)
Changing the form of mouse FOXP2 to include these two human-associated amino acids alters the pitch of these animals’ ultrasonic calls, and affects their degree of inquisitive behaviour. Differences also appear in their neural anatomy. Altering the number of working copies (the genetic ‘dose’) of FOXP2 in mice and birds affects the development of their basal ganglia.
Mice with ‘humanised’ FOXP2 protein show changes in their cortico-basal ganglia circuits along with altered exploratory behaviour and reduced levels of dopamine (a neurotransmitter that affects our emotional responses). So too, human patients with damage to the basal ganglia show reduced levels of initiative and motivation for tasks.
This suggests that FOXP2 is part of a general mechanism that affects our thinking, particularly around our initiative and mental flexibility. These are critical components of human creativity, and are as it happens, essential for our speech.
Basal ganglia circuits process and organise signals from other parts of the brain into sequences. Speaking involves coordinating a complex sequence of muscle actions in the mouth and throat, and synchronising these with the out-breath. We use these same muscles and anatomical structures to breathe, chew and swallow; our ability to coordinate them affects our speech, although this is not their primary role.
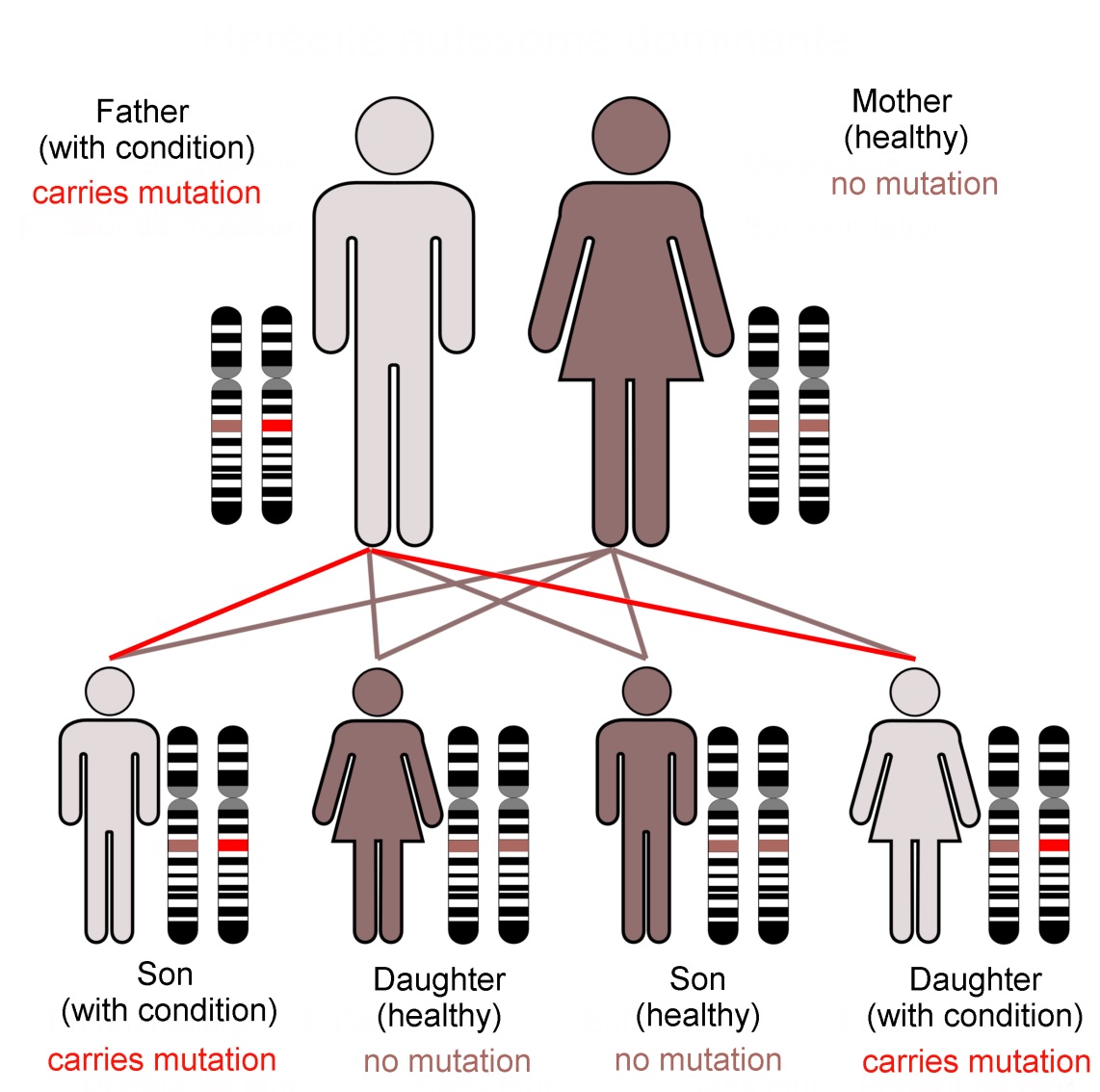
Family KE’s condition, caused by a dominant mutation in the FOXP2 gene, follows an autosomal (not sex-linked) pattern of inheritance, as shown here.Dominant mutations are visible when only one gene copy is present. In... more contrast a recessive trait is not seen in the organism unless both chromosomes of the pair carry the mutant form of the gene. The FOXP2 transcription factor protein is required in precise amounts for normal function of the brain. The loss of one working FOXP2 gene copy reduces this ‘dose’ which is enough to cause the problems that emerged as family KE’s symptoms (Image: Annotated from Wikimedia Commons)
In practice, very few of our 25,000 genes are individually responsible for noticeable characteristics. Most genetically inherited diseases result from the effects of multiple gene loci. FOXP2 is unusual because of its ‘dominant’ genetic character. It does not give us our language abilities, but it is involved in the neural basis of our mental flexibility and agility at controlling the muscles of our mouths, throats and fingers.
In addition, genes are only part of the story of our development. The way we think and subsequently behave alters our emotional state. Feeling stressed or calm affects which circuits are active in our brain. This alters the biochemical state of body organs and tissues, particularly of the immune system, modifying which genes they are using.
The dance between the code stored in our genes and the consequences of our thoughts builds us into what we are mentally, physically and socially. This story is ours to tell. By our experience, and with this genetic vocabulary, we create what we become.
Text copyright © 2015 Mags Leighton. All rights reserved.
References
Chial H (2008) ‘Rare genetic disorders: Learning about genetic disease through gene mapping, SNPs, and microarray data’ Nature Education 1(1):192 http://www.nature.com/scitable/topicpage/rare-genetic-disorders-learning-about-genetic-disease-979
Clovis YM et al. (2012) ‘Convergent repression of Foxp2 3′UTR by miR-9 and miR-132 in embryonic mouse neocortex: implications for radial migration of neurons’ Development 139, 3332-3342.
Enard, W (2011) ‘FOXP2 and the role of cortico-basal ganglia circuits in speech and language evolution’ Current Opinion in Neurobiology 21; 415–424
Enard, W et al (2009) A Humanized Version of Foxp2 Affects Cortico-Basal Ganglia Circuits in Mice Cell 137 (5); 961–971 http://www.sciencedirect.com/science/article/pii/S009286740900378X
Feuk L et at., Absence of a Paternally Inherited FOXP2 Gene in Developmental Verbal Dyspraxia, in The American Journal of Human Genetics, Vol. 79 November 2006, p.965-72.
Fisher SE and Scharff C (2009) ‘FOXP2 as a molecular window into speech and language’ Trends in Genetics 25 (4); 166-177
Lieberman P (2009) ‘FOXP2 and Human Cognition’ Cell 137; 800-803
Marcus GF & Fisher SE (2003) ‘FOXp2 in focus; what can genes tell us about speech and language?’ Trends in Cognitive Sciences 7(6); 257-262
Reimers-Kipping S et al. (2011) ‘Humanised Foxp2 specifically affects cortico-basal ganglia circuits’ Neuroscience 175; 75-84
Scharff C & Haesler S (2005) ‘An evolutionary perspective on Foxp2; strictly for the birds?’ Current opinion in Neurobiology 15:694-703
Vargha-Khadem F et al. (2005) ‘FOXP2 and the neuroanatomy of speech and language’ Nature Reviews Neuroscience 6, 131-138 http://www.nature.com/nrn/journal/v6/n2/full/nrn1605.html
Wapshott N (2013) ‘Martin Luther King's 'I Have A Dream' Speech Changed The World’ Huffington post, 28th August 2013 http://www.huffingtonpost.com/2013/08/28/i-have-a-dream-speech-world_n_3830409.html
Webb DM & Zhang J (2005) ‘Foxp2 in song learning birds and vocal learning mammals’ Journal of Heredity 96(3);212-216